Overall Electrical Effectiveness (OELE) is intended to drive behaviors that ensure the electrical pathways for plant equipment are balanced, aligned, and unrestricted.
Like other proactive metrics featured in this series, OELE is the product of an inventory count to determine the percentage of machines free from common electrical faults, which can compromise the operational reliability of typical plant equipment. Overall Electrical Effectiveness drives up Mean-Time-Between/To-Failure (MTBF/MTTF), which is our goal.
Overall Electrical Effectiveness (OELE) is a leading indicator that drives proactive behaviors, which, in turn, drives asset reliability.
What OELE Is and Why It’s Important
In their various forms, electric motors are the prime movers for most industrial plants. Their reliable and efficient operation is essential to a plant’s operational reliability and profitability.
As mentioned above, the purpose of monitoring OELE is to ensure that our electrical pathways are aligned, balanced across the three phases, and free from excessive resistance/impedance or stray electrical activity.
Overall Electrical Effectiveness is calculated as the product of multiplying the following three factors:
- Percentage of machines where Total-Harmonic-Distortion Targets (THD) are met.
- Percentage of machines that are electrically balanced for voltage, current, resistance, and inductance across the three phases.
- Percentage of machines free from electrical-connector faults, which introduce excessive resistance/impedance, arcing or flashover, tracking, or coronas that cause partial discharge.
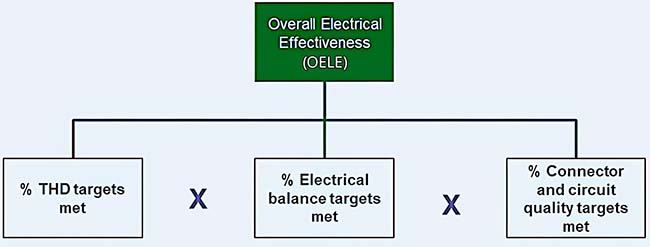
The simple formula for OELE is the product of multiplying the “behavioral inventories” for the three input factors.
Like the previously discussed leading metrics (OIE, OLE, and OVE), we need to consider each of the three OELE components/metrics as an inventory process. We’re simply adding up the complying events and dividing by the total.
1. Percentage of Total-Harmonic-Distortion Targets Met
Harmonic distortion occurs when the electrical supply contains harmonics of the line frequency, which is either 50 Hz or 60 Hz, depending upon your location. In other words, if the line voltage is 460V at 60 Hz frequency, distortion occurs if measured voltage exists at 120 Hz, 180 Hz, 240 Hz, or any other multiple of the line frequency.
The figure below is a highly animated example of a measured voltage of 120V at 180 Hz, the third harmonic. This amount of distortion would not be observed in the plant; it is for illustrative purposes only.
As shown, in a line voltage of 460VAC and a frequency of 60 Hz, a harmonic is observed with 120VAC at 180 Hz (the third harmonic). This amount of distortion would not be observed in a plant. It is used here for illustrative purposes only.
As you can see from the illustration, the third harmonic is out of phase with the line frequency. It distorts the sinusoid, reduces the available voltage to power the motor, and increases the required current. This produces resistive (I2R) losses and generates heat, accelerating the deterioration of winding insulation and other components.
Excessive harmonic distortion reduces available voltage, increases current draw, generates heat, and accelerates motor degradation.
Harmonic distortion is expressed as a percentage and, for any given harmonic, is calculated by dividing the voltage of the harmonic by the voltage at line frequency and then multiplying by 100.
For total harmonic distortion, simply square the voltage for each harmonic, sum them, divide the square root of the sum by the line frequency voltage, and multiply by 100. IEEE 519 calls for specific limits depending upon voltage. For example, motors between 1kv and 69kv should have less than 5% (less is better).
Harmonic distortion can originate from the power supply, be created in the plant, or a combination of both.
2. Percentage of Electrical-Balance Targets Met
Electric motors must be balanced on voltage, current, resistance/impedance, and inductance across the three phases. Let’s start with voltage imbalance. First, calculate the average voltage for all three phases. Then, find the maximum deviation, divide the deviation by the average, and multiply by 100 to get the voltage imbalance percentage.
Any voltage imbalance of more than 2% percent is cause for concern. That’s because it generates heat—lots of heat—since the voltage deficit must be offset by increasing current, which produces I2R losses and electrical heating. For example, a 3% voltage imbalance will increase temperatures by about 20%.
A 5% voltage imbalance results in a whopping 60% increase in temperature. So, a motor that typically runs at 65°C (149°F) could heat up to 104°C (219°F). The winding insulation life would be cut in half for every 10°C (18°F) increase in temperature.
Furthermore, voltage imbalance produces a derate of an electric motor. That means the unit can’t produce the nameplate power. For example, if the nameplate on an electric motor is 100 kW (134 hp), a 5% voltage imbalance would reduce the power capacity to 75 kW (101 hp). If you placed an 80% load on a motor, you would be operating above its derate-adjusted capability.
Voltage imbalance produces a current imbalance. As a rule of thumb, the current imbalance will be about seven times the voltage imbalance—if the voltage imbalance is the cause of the current imbalance. (But that can vary.)
In addition, resistive/impedance imbalance can create a current imbalance. It’s possible to experience a voltage imbalance and a resistive/impedance imbalance that both contribute to a current imbalance. Thus, it makes sense to measure and trend the three conditions.
A 5% voltage imbalance can increase motor temperature by 60%, significantly shortening insulation lifespan and reducing power capacity.
Another element of electrical balance is phase-to-phase inductance. Inductance balance refers to the efficiency with which the electric motor converts electrical energy into mechanical rotating energy, turning the motor’s shaft. An asynchronous electric motor sends electrical energy to the windings in three phases separated by 120 degrees.
The windings are conductive (copper) but separated by insulation. Running current through the stator’s windings creates a magnetic field that induces shaft rotation. If winding quality is poor, the efficiency of the three phases converting electrical energy into mechanical energy will vary.
According to David McKinnon of PDMA, there are no well-defined limits for inductive imbalance, but he suggests no more than 7% for form-wound motors and no more than 12% for loose-wound motors – less is best.
3. Percentage of Electrical-Connector and Circuit-Quality Targets Met
It’s reasonable to say that half of our electrical problems are associated with fastener and connector issues. (In some industries, the percentage is even higher.) These fastener and connector problems include cables to connectors, connectors to terminals, bus bars, fuses and fuse clips, Motor Control Centers (MCCs), bus ducts, and more, i.e., basically any connection point along the electric pathway.
Common problems include, among other things, undersized connections, poorly crimped connections, dirt, grease, or oil contamination, carbonized conductors, and corroded connections (including galvanic corrosion caused by incompatible metals).
Nearly half of electrical failures are traced back to poor connections—underscoring the importance of routine inspections and diagnostics.
We employ infrared thermography (IR) and motor-circuit analysis (MCA) to determine our inventory of complying and noncomplying electrical connections and circuits. I would extend this inventory to include monitoring for arcing/flashover, electrical tracking, and corona/partial discharge. I would also include the dielectric performance of transformer oil, which serves as the insulating medium.
The reliability of electromechanical equipment in the plant requires high-quality power distributed with balanced voltage across circuits that introduce a minimal and balanced resistance/impedance that are properly sized and well connected. The OELE metric can help ensure those requirements are being met.
References
McKinnon, D. (Date Unknown), “Using a Six Fault Zone Approach for Predictive Maintenance on Motors,” PDMA Corp. www.pdma.com/pdfs/Articles/Using_a_Six_Fault_Zone_Approach_for_Predictive_Maintenance_on_Motors.pdf. (Accessed 26 June 2021.)